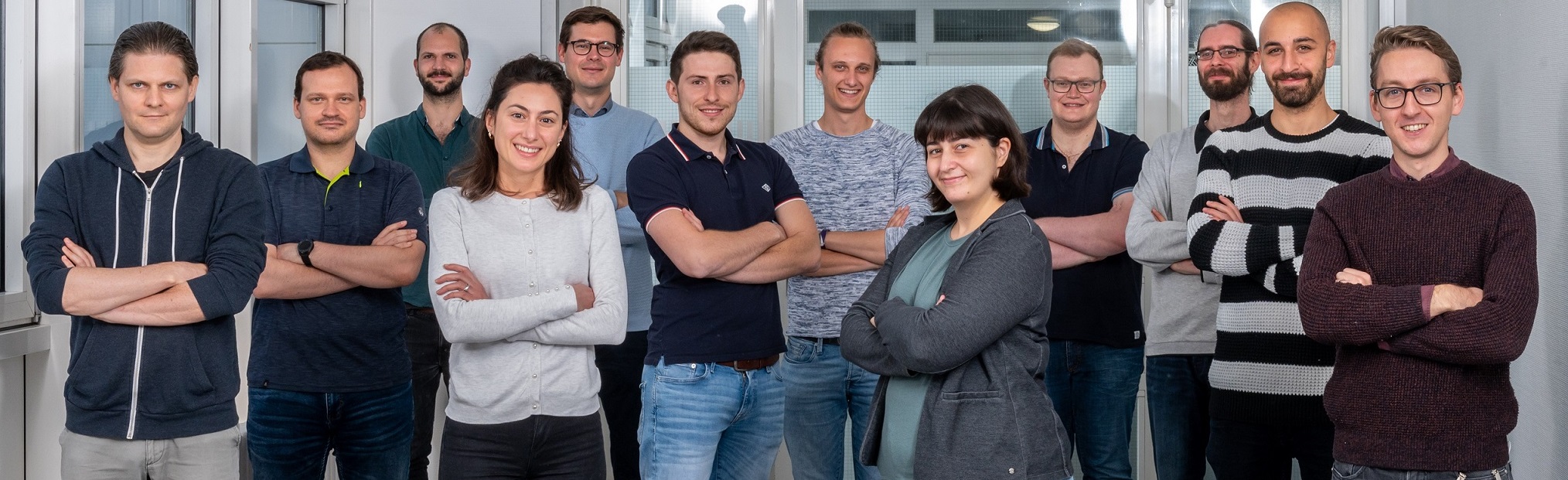
Research Interests
Magnetic resonance imaging biomarkers enable a comprehensive characterization of tissue providing functional, physiological, metabolic, cellular and molecular information beyond anatomical structures. In recent years, hyperpolarization techniques allowed to increase the inherent low sensitivity of magnetic resonance by more than four orders of magnitude, opening new applications ranging from the tracking of chemical reactions in real-time to the direct monitoring of metabolic processes in vivo. Another functional MRI technique that has already shown promise in characterizing tissue in clinical studies is diffusion-weighted MRI (DW-MRI). It enables the quantitative assessment of the apparent diffusion coefficient (ADC) that depends on microstructural tissue properties, including cellularity and intercellular matrix composition. In our group, we focus on the development of sensitive hyperpolarized molecules for imaging metabolism and pH as well as advanced diffusion MRI techniques aiming to provide novel imaging biomarkers to characterize tissue function. We exploit a multimodal approach utilizing the combined strength of high sensitivity PET tracers for simultaneous PET/MRI.
Application - Open Positions
Postdoc positions
Talented and motivated postdoctoral researchers with a strong background in NMR, MRI, hyperpolarization, molecular imaging, or related fields are encouraged to apply to Prof. Schilling directly via e-mail. Please include your CV, list of publications and briefly describe your research interests and project ideas. Postdocs are expected to apply for stipends and fellowships, such as from the Humboldt foundation, the DFG, HFSP or TUM. We will support you in the application process!
PhD positions
There are currently no open positions
Master projects
Motivated undergraduate students interested in joining the group for a semester project, Bachelor’s thesis, or Master’s thesis are encouraged to apply to Prof. Schilling directly via e-mail. Please include your CV and briefly describe your research interests and project ideas.
1. pH Biosensors
Relevant publications:
1. Christian Hundshammer, Martin Grashei, Alexandra Greiner, Steffen J. Glaser, Franz Schilling; ‘pH Dependence of T1 for 13C‐Labelled Small Molecules Commonly Used for Hyperpolarized Magnetic Resonance Imaging’, ChemPhysChem (2019); 20:798-802
2. Simone Köcher, Stephan Düwel, Christian Hundshammer, Steffen J. Glaser, Franz Schilling, Josef Granwehr, Christoph Scheurer; ‘Ab Initio Simulation of pH-Sensitive Biomarkers in Magnetic Resonance Imaging’, The Journal of Physical Chemistry A (2018); 122(40):7983-7990
3. Christian Hundshammer, Stephan Düwel, David Ruseckas, Geoffrey Topping, Piotr Dzien, Christoph Müller, Benedikt Feuerecker, Jan-Bernd Hövener, Axel Haase, Markus Schwaiger, Steffen J. Glaser, Franz Schilling; ‘Hyperpolarized Amino Acid Derivatives as Multivalent Magnetic Resonance pH Sensor Molecules’, Sensors (2018); 18(2):600
4. Stephan Düwel, Christian Hundshammer, Malte Gersch, Benedikt Feuerecker, Katja Steiger, Achim Buck, Axel Walch, Axel Haase, Steffen J. Glaser, Markus Schwaiger and Franz Schilling; ‘Imaging of pH in vivo using hyperpolarized 13C-labeled zymonic acid’, Nature Communications (2017); 8:15126
5. Christian Hundshammer, Stephan Düwel, Simone Köcher, Malte Gersch, Benedikt Feuerecker, Christoph Scheurer, Axel Haase, Steffen J. Glaser, Markus Schwaiger, Franz Schilling; ´Deuteration of hyperpolarized 13C-labelled zymonic acid enables sensitivity-enhanced dynamic MRI of pH´; ChemPhysChem (2017); 18(18):2422-2425 (cover page)
6. Christian Hundshammer, Stephan Düwel, Franz Schilling; ´Imaging of Extracellular pH Using Hyperpolarized Molecules´, Israel Journal of Chemistry (2017); 57(9)788-799
2. Multimodal Metabolic Imaging
Relevant publications:
1. Christoph A. Müller, Christian Hundshammer, Miriam Braeuer, Jason G. Skinner, Stephan Berner, Jochen Leupold, Stephan Düwel, Stephan G. Nekolla, Sven Månsson, Adam E Hansen, Dominik von Elverfeldt, Jan H. Ardenkjaer‐Larsen, Franz Schilling, Markus Schwaiger, Jürgen Hennig, Jan‐Bernd Hövener; ‘Dynamic 2D and 3D mapping of hyperpolarized pyruvate to lactate conversion in vivo with efficient multi‐echo balanced steady‐state free precession at 3 T’, NMR in Biomedicine (2020); in press: https://doi.org/10.1002/nbm.4291
2. Geoffrey J. Topping, Christian Hundshammer, Luca Nagel, Martin Grashei, Maximilian Aigner, Jason G. Skinner, Rolf F. Schulte, Franz Schilling; ‘Acquisition strategies for spatially resolved magnetic resonance detection of hyperpolarized nuclei’, Magnetic Resonance Materials in Physics, Biology and Medicine (2020); 33:221-256
3. Christian Hundshammer, Miriam Braeuer, Christoph Müller, Adam Hansen, Mathias Schillmaier, Stephan Düwel, Benedikt Feuerecker, Steffen Glaser, Axel Haase, Wilko Weichert, Katja Steiger, Jorge Cabello, Franz Schilling, Jan-Bernd Hövener, Andreas Kjaer, Stephan Nekolla, Markus Schwaiger; ‘Simultaneous characterization of tumor cellularity and the Warburg effect with PET, MRI and hyperpolarized 13C-MRSI’, Theranostics (2018); 8(17):4765
3. Diffusion and Exchange
Relevant publications:
1. Mathias Schillmaier, Athanasia Kaika, Franz Schilling; ‚Disentangling intercompartment exchange from restricted diffusion‘, in: ‚Advanced Diffusion Encoding Methods in MRI‘, Royal Society of Chemistry (2020); accepted manuscript
2. Franz Schilling, Susana Ros, De-En Hu, Paula D’Santos, Sarah McGuire, Elizabeth Mannion, Andre A. Neves, Kevin M. Brindle; ‘The urea transporter – a novel substrate-free MRI gene reporter detected using transmembrane water exchange imaging’, Nature Biotechnology (2017); 35:75-80
3. Benedikt Feuerecker, Markus Durst, Michael Michalik, Günter Schneider, Dieter Saur, Marion Menzel, Markus Schwaiger, Franz Schilling; ´Hyperpolarized 13C Diffusion MRS of Co-Polarized Pyruvate and Fumarate to Measure Lactate Export and Necrosis´; Journal of Cancer (2017); 8(15):3078
4. Franz Schilling, Stephan Düwel, Ulrich Köllisch, Markus Durst, Rolf F. Schulte, Steffen J. Glaser, Axel Haase, Angela M. Otto, Marion I. Menzel; ‘Diffusion of hyperpolarized 13C metabolites in tumor cell spheroids using real-time NMR spectroscopy’, NMR in Biomedicine (2013); 26(5):557-568
A complete list of publications can always found here:
https://scholar.google.de/citations?user=4Q4JKIoAAAAJ&hl=de
https://schillinglab.com/publications/
Postdoc. Researchers | Graduate Students | Research Staff | Undergrad. Students | Alumni |
---|---|---|---|---|
Dr. Frits van Heijster +49 (89) 4140 4558 frits.van.heijster@tum.de |
Martin Grashei, M.Sc. +49 (89) 4140 7722 martin.grashei@tum.de(link sends e-mail) |
Dr. Elisabeth Bliemsrieder |
Jakob Wohlhüter +49 (89) 4140 2986 jakob.wohlhueter@tum.de(link sends e-mail) |
Dr. Christian Hundshammer |
Dr. Jason Skinner +49 (89) 4140 2986 jason.skinner@tum.de |
Athanasia Kaika, M.Sc. |
Markus Mittelhäuser +49 (89) 4140 4560 markus.mittelhaeuser@tum.de |
Pascal Wodtke +49 (89) 4140 4558 pascal.wodtke@tum.de |
Dr. Stephan Düwel |
Dr. Geoffrey Topping +49 (89) 4140 7722 Geoffrey.topping@tum.de |
Luca Nagel, M.Sc. |
Hannes Rolbieski +49 (89) 4140 4560 Hannes.rolbieski@tum.de |
Maximilian Aigner, B.Sc. | |
Mathias Schillmaier +49 (89) 4140 4560 mathias.schillmaier@tum.de |
Sandra Sühnel, M.Sc. |
Ryana Fok |
1. ph Biosensors
"Resolving spatiotemporal pH changes non-invasively in vivo using hyperpolarized pH sensor compounds"
Many efforts have been undertaken to develop an imaging method to characterize hypoxic and acidic regions within tumors, namely a quantitative non-invasive pH imaging technique. However, so far, no technique to non-invasively image extracellular pH has been applicable routinely in the clinic. In humans, pH is mainly regulated by the carbon dioxide/bicarbonate buffer within a narrow pH range, in the blood between pH 7.35 and 7.45. Locally, deviations from the systemic pH are often caused by pathologies, such as cancer, inflammation, infection, ischemia, renal failure or pulmonary disease. Our team identified the pH-sensitive molecule [1,5-13C2]zymonic acid (ZA) which we recently reported in a publication in Nature Communications (Figure 1).
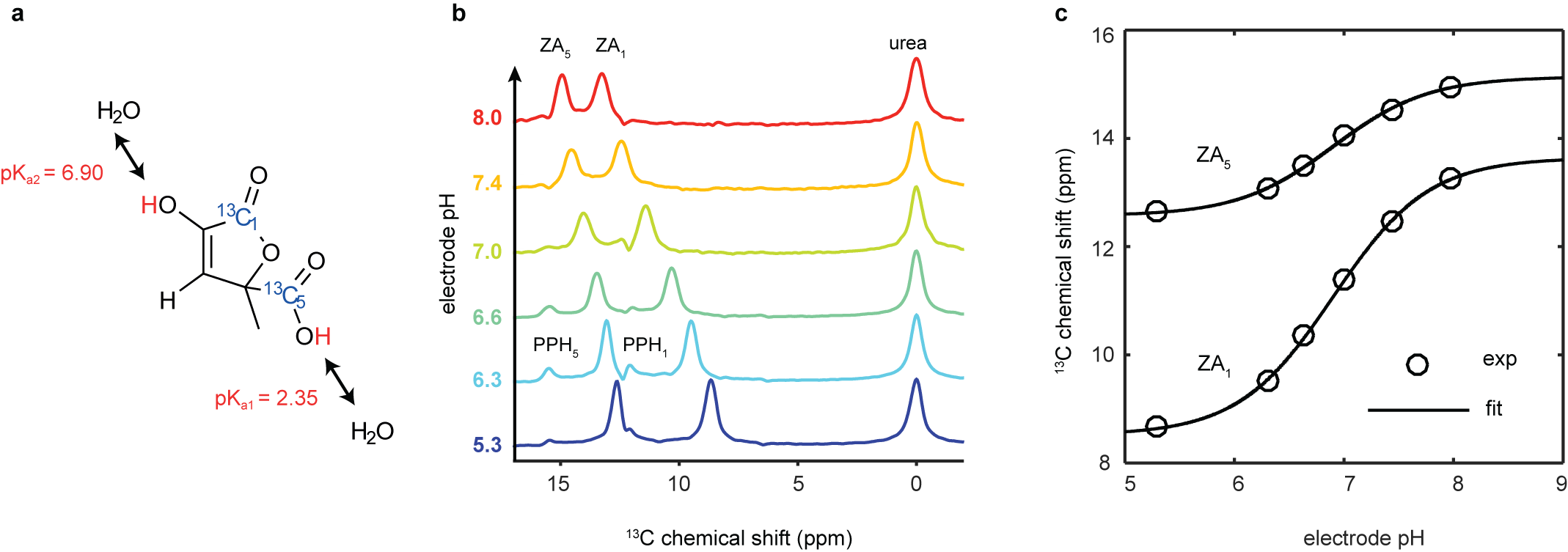
Figure 1. Chemical structure, mechanism and calibration of the pH dependency of ZA at 7 T. a, ZA bears two exchangeable protons, one in a carboxy group with an acid dissociation constant pKa1 = 2.35 and a second one in an enolic hydroxy group with pKa2 = 6.90. b, Spectra of hyperpolarized ZA and urea in buffer phantoms at different pH values. The pH sensitivity in the physiological range is due to pKa2 and results in a change of the chemical shifts of the two 13C-labeled carbon positions with respect to the pH-insensitive 13C-urea peak as a function of pH. Additional peaks of a decay product of ZA, parapyruvate hydrate (PPH), can be identified. c, The fit of the data from (b) results in a calibration curve with a direct dependence of pH on the chemical shift.
To increase the sensitivity in 13C experiments, we developed a hyperpolarization protocol for ZA. Results in vivo in kidneys and tumors (Figure 2) clearly demonstrate that ZA represents a feasible novel candidate for pH imaging with advantages over existing methods. The demonstrated robustness of using ZA for extracellular pH imaging, its lack of toxicity and its accuracy render this new pH imaging technique promising for further development.
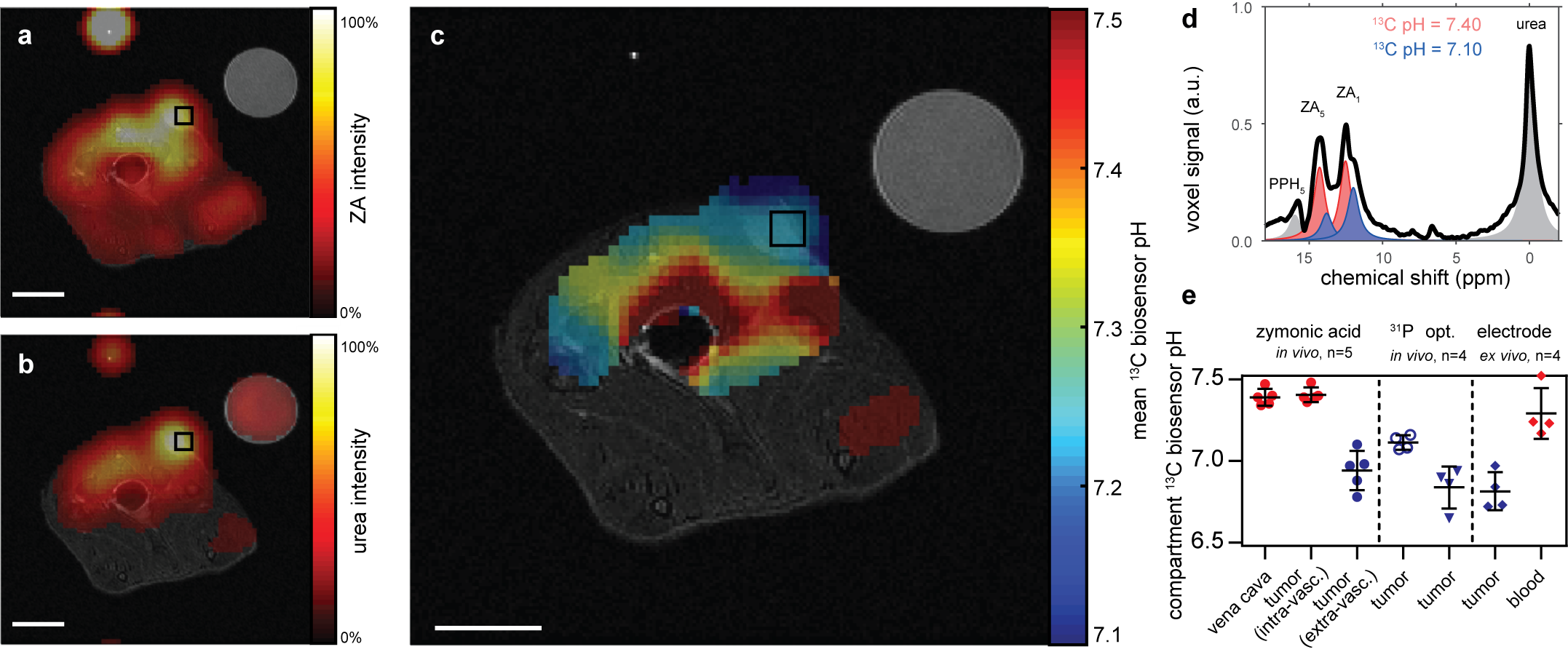
Figure 2: Hyperpolarized ZA in vivo pH measurements show an acidic tumor pH at 7 T. Representative tumor data from a hyperpolarized 13C measurement (colored) in an axial slice overlayed on anatomical proton images (grayscale). A calibration phantom containing 13C urea and the catheter used for injection are visible. Both (a) ZA and (b) urea accumulate in the MAT B III tumor. (c) The mean pH map shows a lower pH value in the tumor compared to the surrounding tissue. For all 13C images, intensity windows are based on sufficiently high signal levels for either (intensity images) or both (pH images) ZA and urea. Spectra from tumor voxels (d), shown for one representative animal) are best fitted with two pairs of ZA peaks (red, blue) and consistently show different pH values in the tumor in comparison to the vena cava, demonstrated in five animals (e) individual datapoints and mean ± s.d.) and compared with three other interstitial/extracellular pH measurements in four animals, namely 31P MRS in vivo using 3-APP, a needle-type optical sensor in vivo and an ex vivo tissue electrode pH measurement. Scale bars, 1 cm.
In another recent study, we show that hyperpolarized and deuterated [1,5-13C2, 3,6,6,6-D4]zymonic acid (ZAd) can be used as an even more sensitive in vivo biosensor to non-invasively image pH. Furthermore, we demonstrate real-time imaging of dynamic pH changes in vitro.
2. Multimodal Metabolic Imaging
Quantifying metabolism in vivo using PET/MRI with hyperpolarized compounds and development of MRI pulse sequences
Magnetic resonance spectroscopic imaging (MRSI) has been used to study biochemical processes non-invasively, although it suffers from a low sensitivity and requires long scan times. The invention of hyperpolarization (HP) techniques has shifted these limits, allowing signal enhancements of 13C-labelled metabolites by more than five orders of magnitude. However, hyperpolarized signals decay with the spin-lattice relaxation time (T1) which is on the order of a few tens of seconds, limiting the time available for signal acquisition and requiring fast signal acquisition.
Hyperpolarized [1-13C]pyruvate is most often used in vivo, because of its key role in glucose metabolism, its fast metabolic conversion to lactate, its high achievable polarization level and a relatively long T1. Tumors readily internalize and metabolize pyruvate to lactate by lactate dehydrogenase (LDH-A). The expression of LDH-A is upregulated in many tumors, which results in an increased pyruvate-to-lactate conversion that can be quantified with MRSI.
We attempt to assess the flux of glucose through the glycolytic pathway simultaneously with both FDG-PET and pyruvate imaging. In a recent study, we established and validated a workflow at a clinical whole-body PET/MR for the multiparametric characterization of the glycolytic flux in a pre-clinical MAT-B-III breast cancer model. We analyzed the in vivo correlation of FDG uptake with pyruvate-to-lactate conversion and performed a longitudinal study with a group of tumor-bearing rats to study the effect of changing tumor cellularity on metabolic PET and MRSI data (see Figure 1).
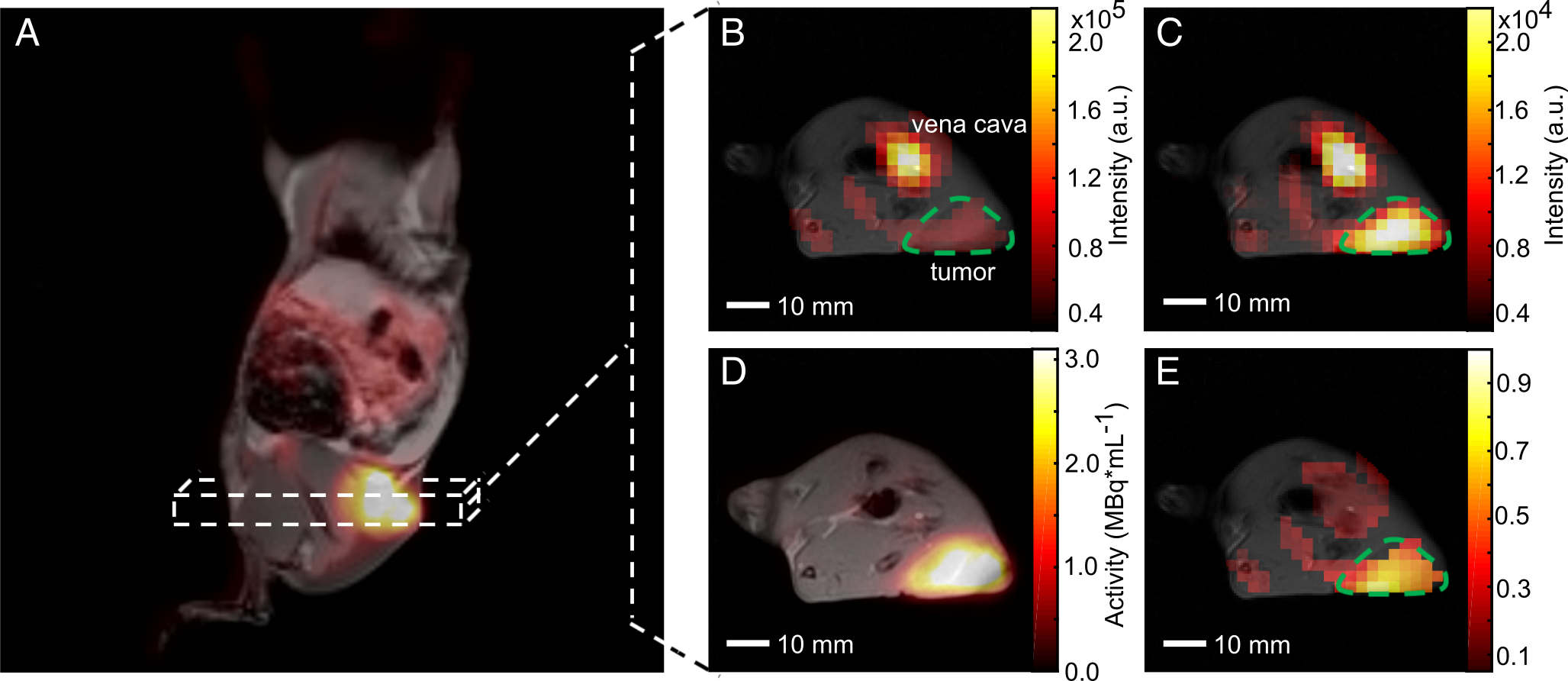
Figure 1. Qualitative comparison of simultaneously imaged FDG uptake and pyruvate-to-lactate exchange in MAT-B-III tumor-bearing rats. (A) Coronal slice of a late frame PET image overlaid on the respective slices of an MPRAGE image. Static axial images of pyruvate (B), lactate (C) and FDG (D) in the same orientation. (E) is the ratio of (C) and (B) showing a higher lactate to pyruvate ratio in the tumor than observed in the surrounding tissue, which correlates well with the high FDG uptake shown in (D). Note that for CSI images, only pixels with a signal > 20% of the maximum signal of hyperpolarized pyruvate and lactate are displayed, respectively. Note further that the pyruvate signal is about a factor of ten higher than that of lactate and that the nominal resolution of 13C-images was two-fold higher than the one of images for quantitative analysis.
In this study, single-frequency bSSFP (without multi-echoes) was used for consecutively imaging individual hyperpolarized metabolites in vitro and in vivo. The Warburg effect was imaged by observing the exchange of [1-13C]pyruvate and [1-13C]lactate. In a recent study, we investigated multi-echo-bSSFP-IDEAL at 3T. Compared to previous results, the spatial and temporal resolution was substantially improved such that observation of the metabolic conversion in one slice or 3D metabolite mapping became possible (see Figure 2).
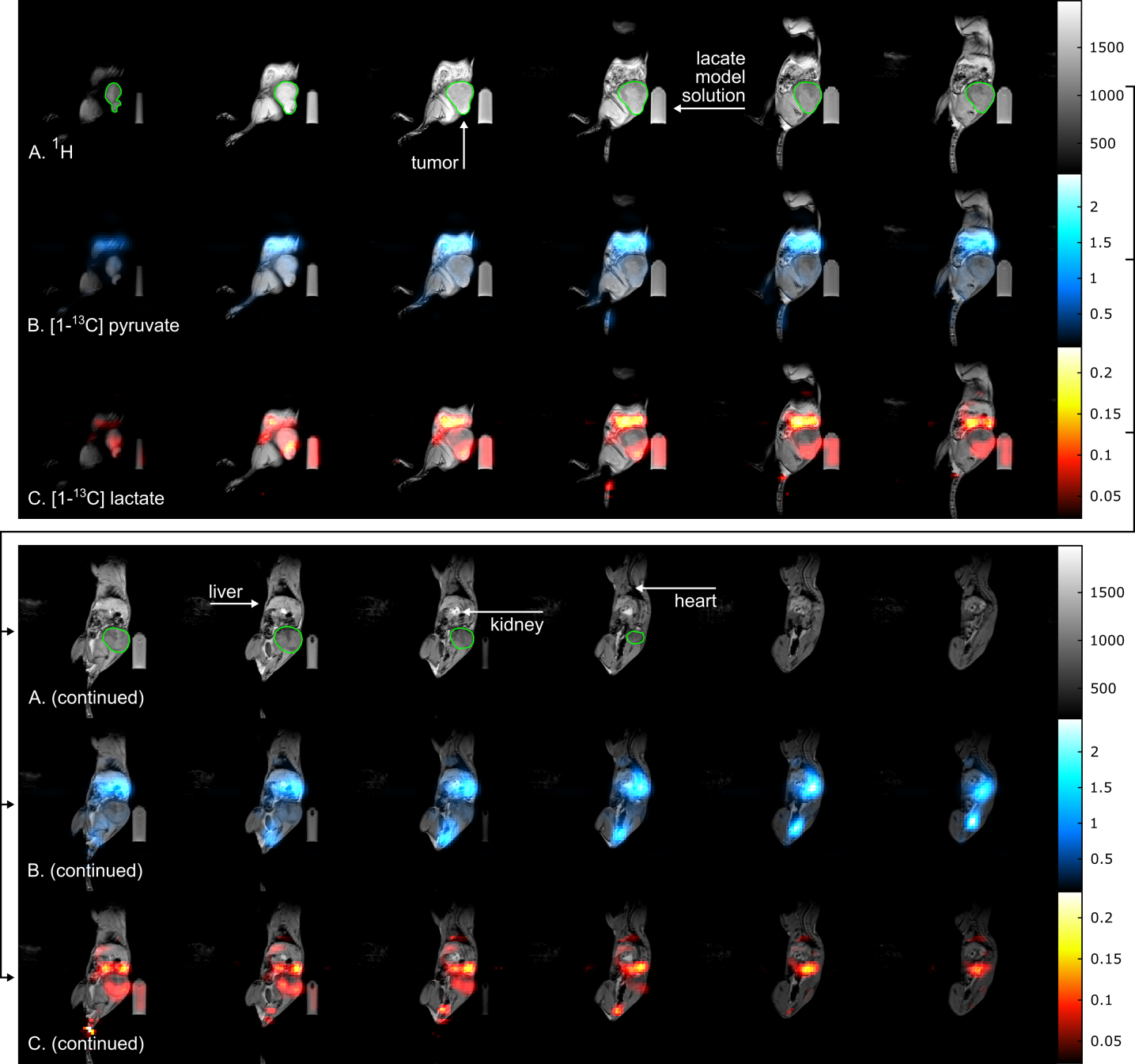
Figure 2: Sagittal slices of a 3D metabolite map. In vivo 1H MRI (A, gray) and 3D 13C metabolite maps of pyruvate (B, blue) and lactate (C, red) of a tumor bearing rat obtained with a 3D me-bSSFP. The scan was started right after injection was finished and ~45 s after dissolution. The injection lasted 26 s and the 3D scan time was 6.8 s. Strong signal of lactate and pyruvate was observed in the stomach, the blood vessels, liver, kidneys and heart (in descending order of signal intensity). The subcutaneous tumor (green outline) exhibited strong and spatially variable lactate signal, which may be a sign of metabolic heterogeneity. Note, that the image color scales start at 2.5% and 10% of the maximum intensity for pyruvate and lactate, respectively. Not all slices of the 3D volume are shown.
Further investigations of sequence variations bSSFP for fast 3D metabolite imaging are currently being investigated within the group.
3. Diffusion and Exchange
Characterizing tissue properties - cellularity and water permeability
Detection of tumor response to treatment in the clinic is largely based on imaging a reduction of tumor size as a marker of treatment response. However, reductions in tumor size may take several weeks and are therefore difficult to detect if a rapid evaluation of a treatment strategy is needed. Therefore, for early detection of tumor response to treatment, novel response markers are to be found. Alternative response markers, e.g. 18F-FDG-uptake, which is reduced in gastrointestinal tumors already 24 h after treatment with Imatinib, have been demonstrated but involve ionizing radiation and thus make repeated exams difficult. Further alternative techniques, such as diffusion-weighted imaging (DWI) have been shown to report on a reduction in tumor cellularity resulting in increased ADCs as a marker for treatment response that precedes reduction in tumor size. Importantly, the MRI contrast being generated using
the diffusion properties of water molecules does not involve exogenous contrast agents. It therefore represents an exquisite non-invasive way to probe the tumor microenvironment using commercially available clinical MRI equipment which renders clinical translation of diffusion-based MRI protocols feasible.
In our group we optimize and develop protocols for probing diffusion microstructure in vivo using DWI for applications in oncology. ADCs and image performance are evaluated using established phantoms for ADC validation and characterisation of image artifacts. The quality of in vivo diffusion-weighted MRI acquisition heavily depends on the reduction of motion artifacts which we want to tackle both by respiratory gating and retrospective artefact reduction.
However, ADC does not always increase with successful treatment progression and is a relatively late marker of cell death only sensitive upon excessive necrosis. Therefore, there is a need for novel IBs to image early necrotic cell death.
Recently, a novel MRI contrast mechanism based on pulsed field gradient magnetic resonance methods has been introduced for in vivo measurements, which detects transmembrane water exchange, quantified by the so-called apparent exchange rate (AXR). The technique named ‘filter-exchange imaging’ (FEXI) relies on a dual diffusion-weighted MRI sequence and has been pioneered by a group of researchers from Lund University. In our recent publication in Nature Biotechnology we showed that it is even sensitive enough to pick up a small amount of ca. 100,000 cells that have been genetically modified to express water channel proteins thereby altering their transmembrane water permeability (Figure 2).
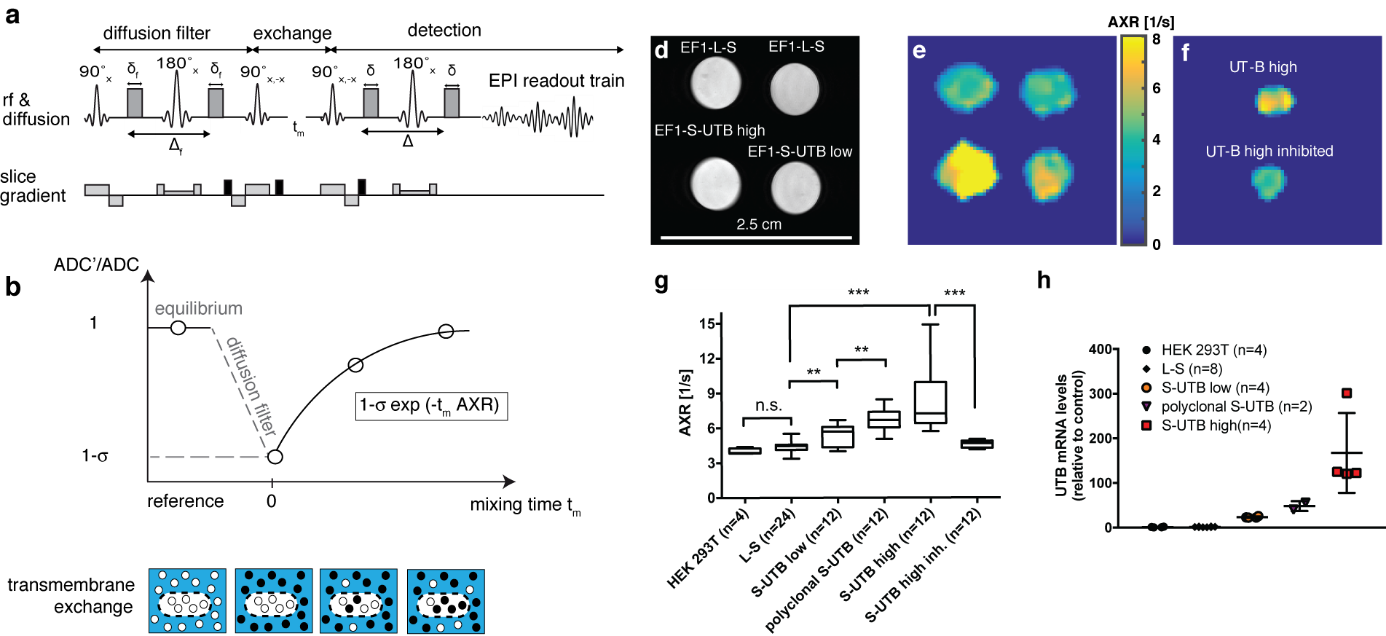
Figure 2. Imaging transmembrane water-exchange. a, The filter-exchange imaging (FEXI) sequence consists of a diffusion filter module, a storage/exchange module, and signal detection using an EPI-readout. b, At equilibrium, with no diffusion filter, a reference ADC is acquired. When the diffusion filter is applied, signal from fast diffusing extracellular water is suppressed, indicated by the black dots, resulting in a different weighting of the signal contributions from the two compartments and leading to a reduction in the measured ADC’. This decrease in ADC is determined by the filter efficiency . With increasing mixing times, tm, between the filter and detection modules, exchange of water between the two compartments results in an increase in the measured ADC. Relaxation back to the equilibrium ADC can be described by the apparent exchange rate constant, AXR. d, Reference proton image of tubes containing dense pellets of the indicated cell lines. e, AXR maps of the cell pellets shown in d). f, AXR map of pellets of EF1-S-UTB high cells. For one of the pellets the cells had been incubated with 170 mM N-N’-Dimethylthiourea. g, Independent experiments show increased AXR with increasing UT-B expression (n = 4-24) and a return to base-level AXR after inhibition of UT-B. h, Real-time PCR measurements of UT-B mRNA expression levels. Data shown in g and h are mean ± SD. Significance was tested using a Mann-Whitney-Wilcoxon test; significance levels: n.s. p > 0.05, * p ≤ 0.05, ** p ≤ 0.01, *** p ≤ 0.001, **** p ≤ 0.0001. Figure adapted from our recent publication in Nature Biotechnology30.
Funding
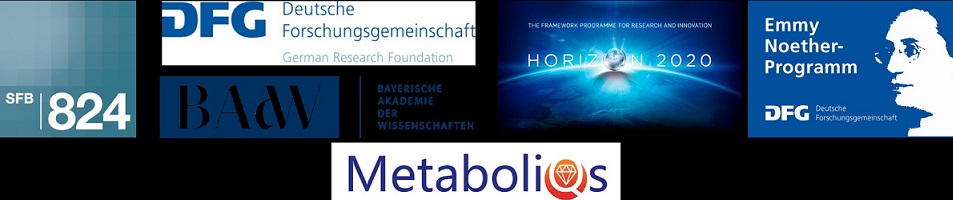
- Deutsche Forschungsgemeinschaft (German Reserach Foundation) - Emmy Noether Programm & SF824
- Horizon2020 (Project MetaboliQs)
- Bayerische Akademie der Wissenschaften (Bavarian Academy of Sciences and Humanities)